A team of researchers from Rice University has uncovered a promising strategy to generate vascular networks, one of the most daunting structures in the human body. Using powdered sugar and selective laser sintering, the researchers were able to build large structures from complex, branching, and intricate sugar networks that dissolve to create pathways for blood in lab-grown tissue.
This is the team’s latest effort to build complex vascular networks for engineered tissues to show that they could keep densely packed cells alive for two weeks. The findings of their study—published in the Nature Biomedical Engineering journal—prove that developing new technologies and materials to mimic and recapitulate the complex hierarchical networks of vessels gets them closer to providing oxygen and nutrients to a sufficient number of cells to get a meaningful long-term therapeutic function.
“One of the biggest hurdles to engineering clinically relevant tissues is packing a large tissue structure with hundreds of millions of living cells,” said study lead author Ian Kinstlinger, a bioengineering graduate student at Rice’s Brown School of Engineering. “Delivering enough oxygen and nutrients to all the cells across that large volume of tissue becomes a monumental challenge. Nature solved this problem through the evolution of complex vascular networks, which weave through our tissues and organs in patterns reminiscent of tree limbs. The vessels simultaneously become smaller in thickness but greater in number as they branch away from a central trunk, allowing oxygen and nutrients to be efficiently delivered to cells throughout the body.”
Overcoming the complications of 3D printing vascularization has remained a critical challenge in tissue engineering for decades, as only a handful of 3D printing processes have come close to mimic the in vivo conditions needed to generate blood vessels. Without them, the future of bioprinted organs and tissues for transplantation will remain elusive. Many organs have uniquely intricate vessels, like the kidney, which is highly vascularized and normally receives a fifth of the cardiac output, or the liver, in charge of receiving over 30% of the blood flow from the heart. By far, kidney transplantation is the most common type of organ transplantation worldwide, followed by transplants of the liver, making it crucial for regenerative medicine experts to tackle vascularization.
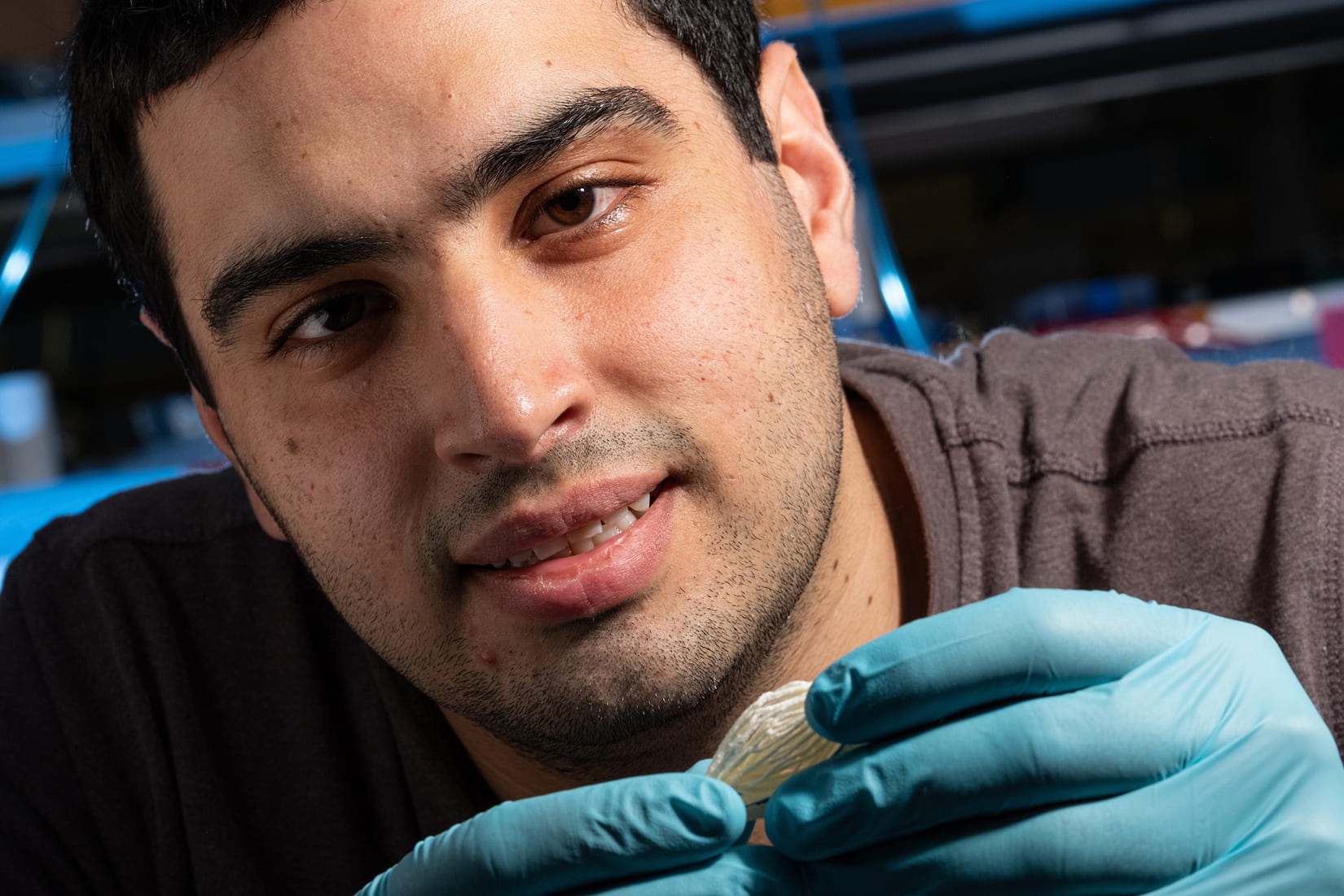
Ian Kinstlinger with a blood vessel template he 3D printed from powdered sugar (Credit: Jeff Fitlow/Rice University)
In the last few years, extrusion-based 3D printing techniques have been developed for vascular tissue engineering, however, the authors of this study considered that the method presented certain challenges, which led them to use a customized open-source, modified laser cutter to 3D print the sugar templates in the lab of study co-author Jordan Miller, an assistant professor of bioengineering at Rice.
Miller began work on the laser-sintering approach shortly after joining Rice in 2013. The 3D printing process fuses minute grains of powder into solid 3D objects, making possible some complex and detailed structures. In contrast to more common extrusion 3D printing, where melted strands of material are deposited through a nozzle, laser sintering works by gently melting and fusing small regions in a packed bed of dry powder. According to Miller, “both extrusion and laser sintering build 3D shapes one 2D layer at a time, but the laser method enables the generation of structures that would otherwise be prone to collapse if extruded.”
“There are certain architectures—such as overhanging structures, branched networks and multivascular networks—which you really can’t do well with extrusion printing,” said Miller, who demonstrated the concept of sugar templating with a 3D extrusion printer during his postdoctoral studies at the University of Pennsylvania. “Selective laser sintering gives us far more control in all three dimensions, allowing us to easily access complex topologies while still preserving the utility of the sugar material.”

Assistant professor of bioengineering at Rice University, Jordan Miller (Credit: Jeff Fitlow/Rice University)
Generating new 3D printing processes and biomaterials for vascularization is among the top priorities for the researchers at Miller’s Bioengineering Lab at Rice. The lab has a rich history of using sugar to construct vascular network templates. Miller has described in the past how sugar is biocompatible with the human body, structurally strong, and overall, a great material that could be 3D printed in the shape of blood vessel networks. His original inspiration for the project was an intricate dessert, even going as far as suggesting that “the 3D printing process we developed here is like making a very precise creme brulee.”
To make tissues, Kinstlinger chose a special blend of sugars to print the templates and then filled the volume around the printed sugar network with a mixture of cells in a liquid gel. Within minutes, the gel became semisolid and the sugar dissolved and flushed away to leave an open passageway for nutrients and oxygen. Clearly, sugar was a great choice for the team, providing an opportunity to create blood vessel templates because it is durable when dry, and it rapidly dissolves in water without damaging nearby cells.
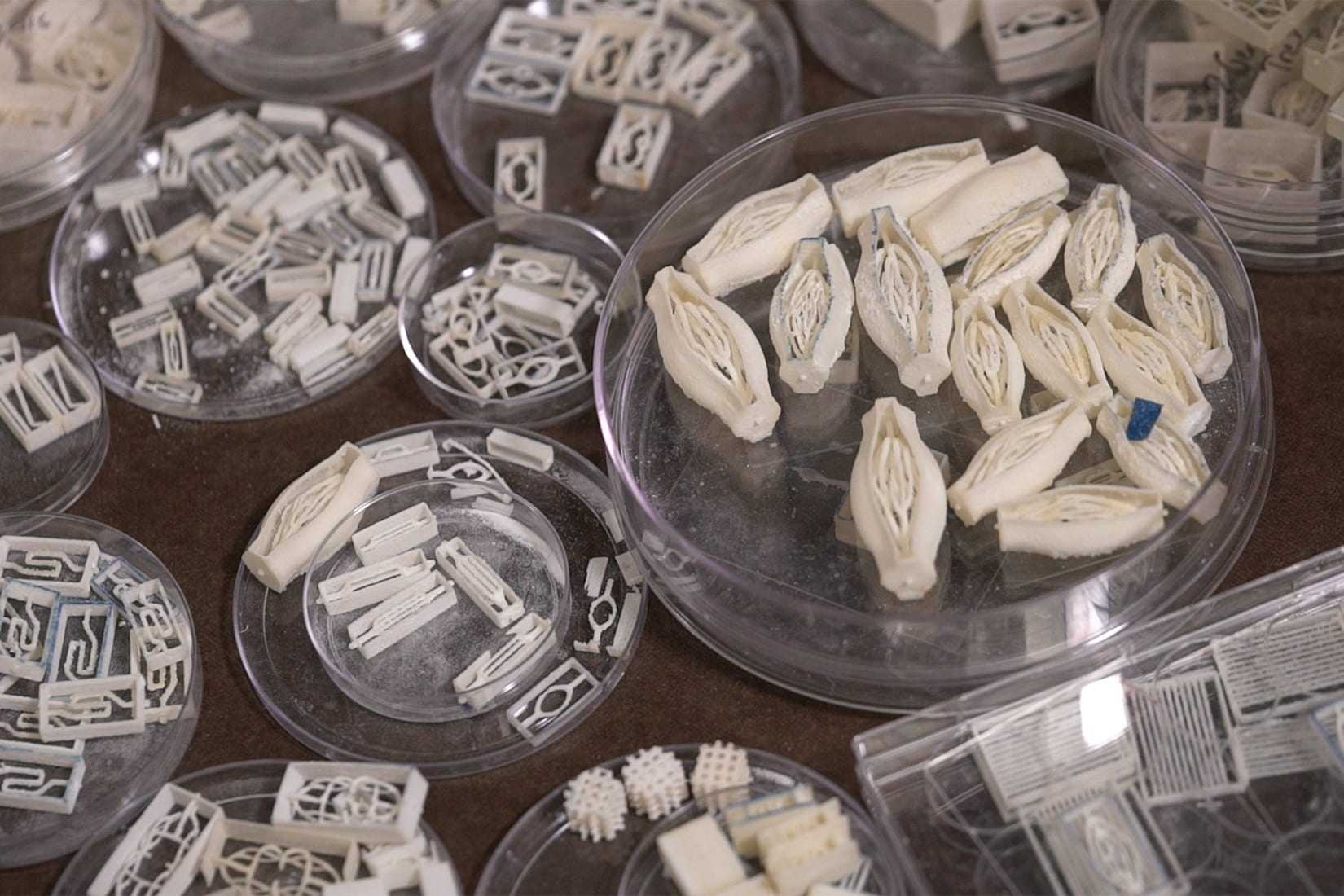
A sample of blood vessel templates that Rice University bioengineers 3D printed using a special blend of powdered sugars. (Credit: B. Martin/Rice University)
In order to create the treelike vascular architectures in the study, the researchers developed a computational algorithm in collaboration with Nervous System, a design studio that uses computer simulation to make unique art, jewelry, and housewares that are inspired by patterns found in nature. After creating tissues patterned with these computationally generated vascular architectures, the team demonstrated the seeding of endothelial cells inside the channels and focused on studying the survival and function of cells grown in the surrounding tissue, which included rodent liver cells called hepatocytes.
The hepatocyte experiments were conducted in collaboration with the University of Washington (UW)’s bioengineer and study co-author Kelly Stevens, whose research group specializes in studying these delicate cells, which are notoriously difficult to maintain outside the body.
“This method could be used with a much wider range of material cocktails than many other bioprinting technologies. This makes it incredibly versatile,” explained Stevens, an assistant professor of bioengineering in the UW College of Engineering, assistant professor of pathology in the UW School of Medicine and an investigator at the UW Medicine Institute for Stem Cell and Regenerative Medicine.
The results from the study allowed the team to continue their work towards creating translationally relevant engineered tissue. Using sugar as a special ingredient and selective laser sintering techniques could help advance the field towards mimicking the function of vascular networks in the body, to finally deliver enough oxygen and nutrients to all the cells across a large volume of tissue.
Miller considered that along with the team they were able to prove that “perfusion through 3D vascular networks allows us to sustain these large liverlike tissues. While there are still long-standing challenges associated with maintaining hepatocyte function, the ability to both generate large volumes of tissue and sustain the cells in those volumes for sufficient time to assess their function is an exciting step forward.”
The post Rice Researchers 3D Print with Lasers and Sugar to Build Complex Vascular Networks appeared first on 3DPrint.com | The Voice of 3D Printing / Additive Manufacturing.