Fluicell is Preparing to be the Next Big Player in Sweden’s Bioprinting Field
Creating innovative tools and high-tech systems for life science researchers around the globe has turned up some fascinating new companies in the last few years; and with Europe currently housing over 35% of biotechnology companies worldwide, we can expect some enticing new discoveries to come. Sweden is certainly not lagging behind, with a buoyant environment for university researchers and students, as well as being known as one of the so-called ‘ideal’ places to hatch startups, one company is quickly breaking new ground. Founded in 2012 as a spin-off from Chalmers University of Technology, Fluicell is a publicly-traded biotech company providing platforms to investigate cell behavior like never before. Using open-volume microfluidics, they wanr to revolutionize how cells are bioprinted.
As a further development to their existing product portfolio, the company has developed a unique high-resolution bioprinting technology in both 2D and 3D called Biopixlar, capable of creating complex tissue-like structures where positioning of individual cells can be controlled from a gamepad, just like you would a videogame. Their original approach is part of a more market-oriented strategy, which brings revolutionary technology straight to the fingertips of users. To get a better sense of what the company is trying to accomplish, 3DPrint.com spoke to Victoire Viannay, Fluicell’s CEO since 2017.
“Since microfluidics is so complex we are trying to create very easy to use platforms for our clients in the life sciences. Our original idea with the Biopixlar was: how to make the system easy to use and fun? So now you can see that we have even incorporated the gamepad, which is a way of creating an easy to use interface,” said Viannay.
Biopixlar uses microfluidics which allows for better control of the material at a micro level due to the precision of a pump or microfluidic tube when it comes to directing the flow of biomaterial to actual printing execution. Having such a precise control at the microlevel, systems naturally scale up to the macrolevel and result in high-resolution prints. Additionally, the technology allows the creation of multi-material prints for bioprinting purposes, with users being able to create the materials within the printer technology itself, avoiding the need for laboratory fabrication of the material. A microfluidic chamber can control the mixing of various materials in house. Resulting in a 3D printed structure that is immediately complete without having to deal with gels or scaffolds.
“We want to be as true as possible to the science, so it is important for us to protect the landscape, and for that we have a good internal team for harnessing and developing knowledge, knowing that we need to have both invention and method patents.”
Fluicell currently has three products on the market, and are now looking actively for partners for the Biopixlar in both Europe and the United States. The research tools Biopen and Dynaflow, allow researchers to investigate the effects of drugs on individual cells at a unique level of detail, as part of their mission to redefine the approach to cell biology, and drug discovery by providing miniaturized instrumentation for single-cell investigations. The company holds a strong IP and patent position with four approved patents in the estate.
Since 2012, the company has moved from Chalmers and established their own laboratories just a few minutes away from the campus, in Gothenburg. There they have commercialized a product portfolio to study single cells, (primarily in the field of drug development), gone public, and launched Biopixlar. Funded by Almi Invest, a local early-stage investor, their aim now is to keep providing innovative tools redefining approaches within cell biology, bioprinting, and secondary drug screening and discovery.
“When the company was created we started at Chalmers, but at some point we thought we had to become more independent from the university, so we came up with our own facilities and discovery team, people who work on tissue and disease models in house so that we can do primary research ourselves and the discovery aspects as a way of helping potential clients discover applications which could benefit their needs. We have this both as a demonstration, and also as a contract research organization (CRO) service.”
With 20 employees, the company is looking to become the next Swedish bioprinting success, after another company born out of the same city as Fluicell, began selling their popular bioprinters and bioinks, that’s Erik Gatenholm’s CELLINK, now a global big player in biotech. Actually, Viannay claims that Sweden is a great country to start a company, just behind the captivating and successful landscape in the United States.
“Sweden is very supportive of new companies. The whole country is built upon innovation, proving that its people were never afraid to try out new things, so it should be the same with bioprinting. Right now there is a very good landscape to work on our projects and i really think that Sweden is ready to support more bioprinting initiatives,” suggested Viannay, who is originally French and moved to Sweden after meeting her husband. She has proved to be a great match for the company because of her strong background in law. With a PhD in the field from the Université Paris II Panthéon/Assas and over more than 10 years of experience in labor laws, human resources and legal management, particularly in the field of scientific research, her incorporation came in at just the right time. Her knowledge came in handy during the company’s IPO in early 2018.
“Fluicell has a good growth model based on market penetration, acquiring new geographic areas and expansion and market diversification. So it has worked very well for us while growing the company, next we would be interested in being a profitable company that is very well recognized in the world thanks to our products, which began with the Biopen, and had great traction among our customers. For our Biopixlar technology we would like to further target other areas, such as regenerative medicine, moving towards building tissues and taking it outside of pure research and development by using it to develop something that can go into regenerative or therapeutic medicine.”
[Image credit: Fluicell]
The post Fluicell is Preparing to be the Next Big Player in Sweden’s Bioprinting Field appeared first on 3DPrint.com | The Voice of 3D Printing / Additive Manufacturing.
SUTD researchers integrate functional components to 3D printed microfluidic devices
UTK: Doctoral Student Explores the Intersection of 3D Printing, Microfluidics & Bioprinting
University of Tennessee at Knoxville Doctoral student, Peter Golden Shankles, presents his dissertation on ‘Interfacing to Biological Systems Using Microfluidics,’ discussing the popular new field of microfluidics and the 3D printed tools that are propelling it forward—for this project and numerous others recently too. 3D printing allows for much greater self-sustainability in the lab as researchers can create tools for experiments and chemical reactions on their own, but attention must also be paid to how such technologies affect chemical transformations and influence biological systems.
As microfluidics are used more frequently with cell-free protein synthesis systems (CFPS), researchers usually set up tubes to experiment with reactions. As the author points out, this is usually easy, but other studies have shown better success with ‘engineered reaction hardware.’ In this study, the researchers aim to begin using microfluidics as well as nanoscale membranes to lessen distances in diffusion and maintain reactions longer with better addition of microfluidics and nanoscale membranes. Success in such research could make impacts in applications such as medicine, when mixing doses of medicine.
Researchers involved in microfluidics have begun to use 3D printing due to the ease in creating components, quickly, and with exponential reduction in production cost. Availability of 3D printers has made a big difference, along with the affordability quotient, but more improvements must be made. FDM 3D printing has also been behind the creation of devices without the need for molds. 3D printed microfluidics are also popular mechanisms in bioprinting today, with the use of hydrogels used in tissue engineering.

3D microfluidics. Using 3D capabilities of the feature-based software, bridges were printed to create an overlapping design with three channels from an offset (a) and side (b) view. (d)Top view – overlapping channels remain separate from one another. (c) Side view – the bridging structure raises off the plane of the glass slide. The expanded view shows the printing direction for the bridging structures. (e) The microfabricated structure along with an inset of the chambers with each channel independent of one another. (f) Shows 3D printed structures connecting channels and overlapping to simplify the device control.
“The field of 3D printed microfluidics is growing to the point that substantial biological discoveries have been made with printed devices along with providing a path to commercialization for microfluidics that is simpler than PDMS based approaches,” says Shankles. “Most plastics used in 3D printing can be injection molded, providing a more straightforward road to commercialization than other techniques.
“Within microfluidics there are a plethora of techniques available to scientists and engineers so that the focus can be returned to biology and answering questions that are out of reach for traditional techniques.”
Shankles makes the obvious point that researchers should understand the proper ‘aspect of nature’ to copy for success in microfluidic platforms, along with realizing that current assays often require more than interactions with just one measurement of density or biochemical activity—and even the most basic systems may require more complicated setup than initially realized; however, Shankles does see the new 3D printing breakthroughs as offering potential for the future.
“The platform introduced here provides a route to expanding co-culture studies and building upon genomic studies to verify proposed interactions in a tractable way that preserves quorum sensing 70 behaviors while providing resources to more deeply understand the effects of chemical communication between species and communities,” concluded Shankles.
While also offering countless innovations to the world, 3D printing also allows scientists to make a wide range of tools—changing the scope of project management today as they as able to create necessary components for the lab themselves such as micro and millifluidic devices, flow chemistry equipment, and microreactors for photocatalysts. Find out more here about how scientists today are interfacing to biological systems with the help of 3D printing. What do you think of this news? Let us know your thoughts! Join the discussion of this and other 3D printing topics at 3DPrintBoard.com.

Signal switching the culture chamber with imbalanced support channel flow rates. (a) The relative flow rate is changed between the fluorescein and buffer support channels every 30 min. (b) shows a detailed version of one transition from the buffer support channel to the fluorescein support channel. (c) epifluorescent micrographs of the culture chamber in the beginning of a transition period at 0, 1, and 5 min.
[Source / Images: Interfacing to Biological Systems Using Microfluidics]
Lawrence Berkeley National Laboratory: Researchers Use Liquid-in-Liquid Printing to Create 3D Fluidic Devices
The mixing of oil and water is generally something most of us have no use for, with the two known to be immiscible liquids—meaning they do not combine together and eventually separate into layers. Researchers at Lawrence Berkeley National Laboratory are currently studying how these types of mixtures could be helpful in a variety of different scientific applications though. Their findings are discussed in a recently published paper, ‘Harnessing liquid-in-liquid printing and micropatterned substrates to fabricate 3-dimensional all-liquid fluidic devices,’ authored by Wenqian Feng, Yu Chai, Joe Forth, Paul D. Ashby, Thomas P. Russell, and Brett A. Helms.
In harnessing liquid structures to create 3D fluidic devices, the researchers explained that such an exercise is an ‘emerging design paradigm’ for chemists today interested in manipulating soft matter and figuring out ways to produce them on demand.
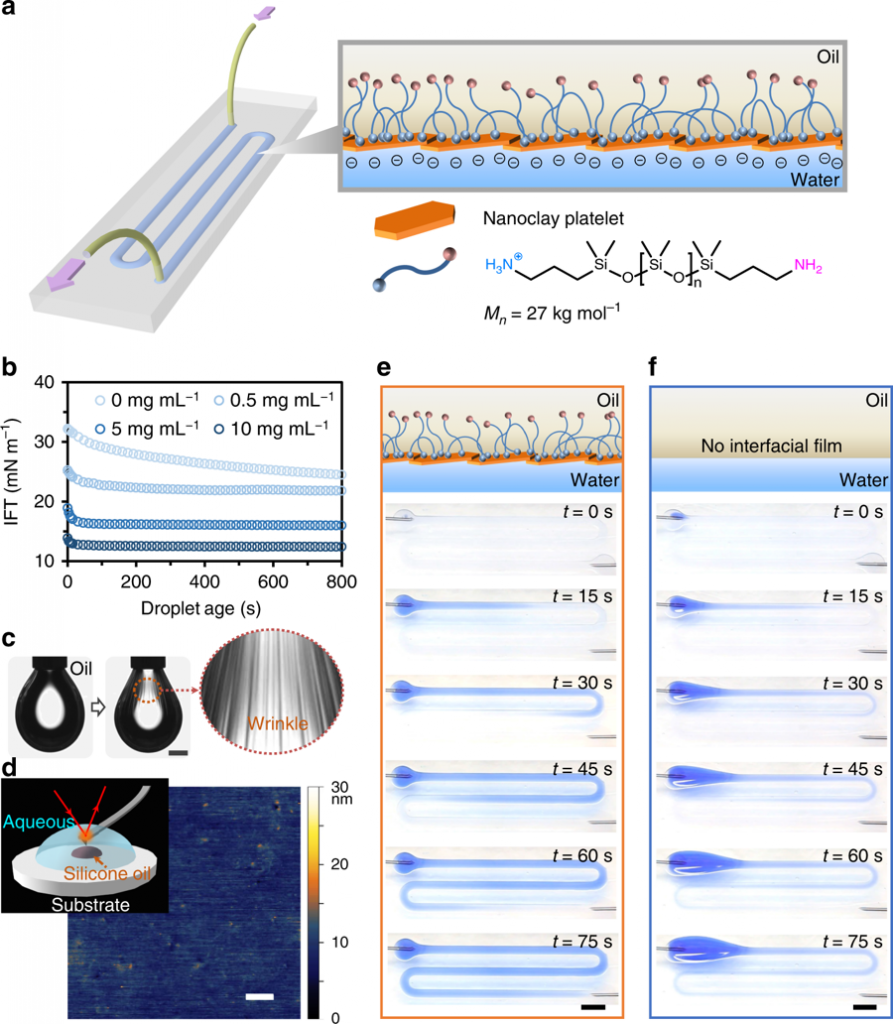
All-liquid fluidic devices stabilized by NPS membranes. a Schematic of an all-liquid fluidic device comprised of immiscible liquid phases confined in space using superhydrophobic−superhydrophilic micropatterned substrates. Nanoclay–polymer surfactants (NPSs) self-assemble at the liquid–liquid interface, forming an elastic wall that allows the all-liquid architecture to maintain integrity while fluid is pumped through the channel. b Temporal evolution of interfacial tension (IFT) of aqueous nanoclay dispersions (0.5, 5, and 10 mg mL−1, pH 7.0) introduced to solutions of H2N-PDMS-NH2 in toluene (10% w/w), illustrating control over the rate of NPS assembly at the interface. c Buckling behavior observed when retracting a droplet cladded with the interfacial NPS film. Scale bar, 1 mm. d In-situ AFM image of the NPS film. The inset shows the schematic diagram of the experimental setup for AFM measurements. Scale bar, 100 nm. e, f Time-lapse images of a solution of blue-colored dye being pumped (10 mL h−1) through the channel in the presence (e) and in the absence (f) of the NPS film. Scale bars, 5 mm. AFM atomic force microscopy
“Still nascent in their development, structured liquids presently lack clear design rules for controlling their 2D or 3D architectures, spatially directing functional components within each liquid phase, and coupling physiochemical processes across the liquid−liquid interface so as to create autonomous chemical systems capable of performing useful work, processing information, or executing logical functions,” state the scientists.
To make such devices, glass supports were coated with superhydrophobic polymers. Next, the researchers used photo-patterning with superhydrophilic channel architectures, also accompanied by an aqueous dispersion of anionic 2D nanoclays.
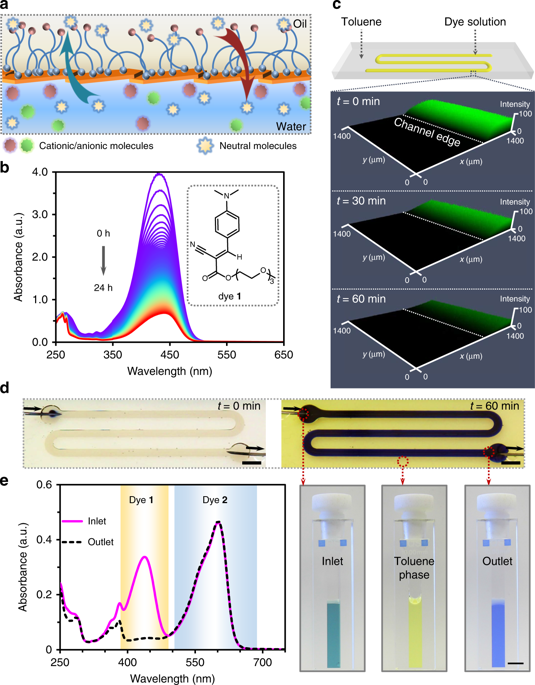
Semi-permeability of 2D NPS interfacial films. a Schematic showing selective mass transfer across the water–oil interface through the NPS film. b Stacked UV–Vis spectra of a neutral dye (1) at 5-min time-intervals, monitoring dye transfer from water to toluene across the NPS membrane film in a masked cuvette. The inset shows the chemical structure of dye 1 engaged in mass transfer. c LSCM images of the fluidic channel after infusing dye 1 solution in the preformed liquid channel covered by toluene. d A fluidic device depicting the flow of a mixed solution of dye 1 and resazurin sodium (2) for chemical separation. [1] = 1.4 mg mL−1, [2] = 1 mg mL−1. Photographs of the diluted inlet, outlet, and overlay of toluene solutions collected in cuvettes show the dye separation after infusing the mixed solution at a flow rate of 0.2 mL h−1 for 1 h. Scale bar, 5 mm. e UV–Vis spectra of the diluted inlet and outlet solutions used to quantify the efficiency of dye 1 partitioning from the aqueous phase to the toluene phase. NPS nanoparticle−polymer surfactant, LSCM laser scanning confocal microscopy
“Interfacial forces are effective in pinning and confining the aqueous phase in arbitrarily complex geometries and a wide range of channel widths,” stated the research team.
The manipulated systems are able to reach the desired steady state quickly, and nanoparticle−polymer surfactants (NPS) topography was presented after the researchers employed atomic force microscopy. They reported that their data demonstrated ‘well-packed nanoclays’ at the interface with no structural problems. The researchers also noted that only microchannels with NPS walls could guide flow at the desired rate. Without them, the aqueous phase proceeded to build up at the channel entrance. The team also noted that maximum flow is completely reliant on the channel’s cross-section and overall architecture.
The team also went on to investigate membrane permeability, functionalization, and further chemical transformations regarding the fluidic devices with the requisite NPS walls. With the use of NPS films, they found that added microchannels were ‘straightforward to introduce’ direct-write methods.
“To contextualize this advance, without the aid of micropatterned substrates and NPS films, constructing channel-like aqueous threads in oil is not typically possible, as the thermodynamic driving force required to reduce interfacial area breaks up aqueous threads into droplets,” stated the researchers.

a Schematic of fabricating 3D microchannels stabilized by nanoclay-surfactant interfacial assembly to connect the separated microchannels by printing of water in oil. Disconnecting these microchannels is also possible by cutting these “bridges” off. b Adjacent channels on a patterned substrate connected by a 3D printed overpass. Na-fluorescein (0.1 mg mL−1) has been added to the clay dispersion to clarify features of the constructs. Scale bar: 2 mm. c Fabrication of a 3D bridge linking separate channels (middle panel) and clipping the bridge (right panel). Scale bar: 500 µm.
The fabricated microchannels offered such a stable structure during research and evaluation that the researchers found they could use them as bridges either connecting separate regions on the substrate, or in connecting the device to an exogeneous entity.
“Our studies uncover a latent learning ability in such devices, in that physiochemical sensing or detection of channel properties and contents can be used to direct the architecture of the device to achieve a specific outcome. Maturation of the design concept led to devices that can execute complex tasks in a logical manner by reversible compartmentalization of function and direction of chemo-energetic flows that operate far from equilibrium conditions,” concluded the researchers.
“The potential for this system to exhibit autonomous learning is evident. Such devices may also be arrayed to generate deep or dark data for machine learning, e.g., from all-liquid (bio)chemical transformations and screens, to build knowledge and understanding from chemical logic.”
Fabrications methods used in 3D design and 3D printing today span many different industries and intricate applications, but you may be surprised to explore further into the science of materials, hardware, and software, and realize how many chemists are using the technology from use in continuous flow systems to studies centered around miniaturization and complex designs in microfluidics. Learn more about current processes in liquid-in-liquid printing here.
What do you think of this news? Let us know your thoughts! Join the discussion of this and other 3D printing topics at 3DPrintBoard.com.
[Source / Images: Harnessing liquid-in-liquid printing and micropatterned substrates to fabricate 3-dimensional all-liquid fluidic devices]

All-liquid microreactors. a–c Schematic of the anchoring of cationic molecules (a), enzymes (b), and nanocrystals (c) to the anionic NPS film lining the microchannels. d, e 3D reconstitution of confocal images of methylene blue (d) and LRSC-labeled HRP (e) to determine the adhesion of cationic species to the NPS wall of the microchannel. Scale bars, 100 µm. f In-situ AFM image of the NPS film immobilized with CTAB-coated Pt nanocrystals at the water−silicone oil interface. Scale bar, 100 nm. g, h Oxidation of TMB (g) and 4-AAP/phenol (h) substrates catalyzed by immobilized HRP in the channels, which generate products with distinctive colors under flow. Scale bars, 5 mm. NPS nanoparticle−polymer surfactant, LRSC lissamine rhodamine B sulfonyl chloride, HRP Horseradish peroxidase, TMB 3,3′,5,5′-tetramethylbenzidine, 4-AAP 4-aminoantipyri
3D Printed Microfluidic Device Designed to Customize Cancer Treatment
Testing cancer treatments is a lot of trial and error currently, and patients are often subject to multiple uncomfortable and time-consuming therapies before finding one that works. Developments have been made, including growing artificial tumors to test drugs on specific cancer types, but these tumors can take weeks to grow and they don’t account for patients’ individual biological makeup. Now, however, researchers from MIT and Draper University have come up with a new option: a 3D printed microfluidic device that simulates cancer treatments on biopsied cancerous tissue.
The device is a chip slightly larger than a quarter that can be 3D printed in about an hour. It has three cylindrical chimneys protruding from the surface, which are ports that input and drain fluids as well as remove unwanted air bubbles. The biopsied tumor fragments are placed in a chamber connected to a network of deliver fluids to the tissue. These fluids could contain things like immunotherapy agents or immune cells. Clinicians can then use imaging techniques to see how the tissue responds to the treatments.
The researchers used a new type of biocompatible resin, traditionally used for dental applications, that can support the long-term survival of biopsied tissue. This contrasts with other 3D printed microfluidic drug testing devices, which have chemicals in the resin that quickly kills the cells. Fluorescence microscopy images showed that the new device, called a tumor analysis platform or TAP, kept more than 90 percent of the tissue alive for at least 72 hours and potentially much longer.
The TAP is cheap and easy to fabricate, so it could quickly be implemented into clinical settings, according to the researchers. The devices is adaptable as well – doctors could 3D print a multiplexed device that could support multiple tumor samples in parallel, so that the interactions between tumor fragments and several different drugs could be modeled simultaneously for a single patient.
“People anywhere in the world could print our design. You can envision a future where your doctor will have a 3-D printer and can print out the devices as needed,” said Luis Fernando Velásquez-García, a researcher in the Microsystems Technology Laboratories. “If someone has cancer, you can take a bit of tissue in our device, and keep the tumor alive, to run multiple tests in parallel and figure out what would work best with the patient’s biological makeup. And then implement that treatment in the patient.”
One potential application is testing immunotherapy, a new treatment method that uses drugs to “rev up” a patient’s immune system to help it fight cancer.
“Immunotherapy treatments have been specifically developed to target molecular markers found on the surface of cancer cells,” said graduate researcher Ashley Beckwith. “This helps to ensure that the treatment elicits an attack on the cancer directly while limiting negative impacts on healthy tissue. However, every individual’s cancer expresses a unique array of surface molecules — as such, it can be difficult to predict who will respond to which treatment. Our device uses the actual tissue of the person, so is a perfect fit for immunotherapy.”
The research was published in a paper entitled “Monolithic, 3D-Printed Microfluidic Platform for Recapitulation of Dynamic Tumor Microenvironments.“
“A key challenge in cancer research has been the development of tumor microenvironments that simulate mechanisms of cancer progression and the tumor-killing effects of novel therapeutics,” said Jeffrey T. Borenstein, who leads the immuno-oncology program at Draper. “Through this collaboration with Luis and the MTL, we are able to benefit from their great expertise in additive manufacturing technologies and materials science for extremely rapid design cycles in building and testing these systems.”
Microfluidic devices are typically produced via micromolding with PDMS. The technique was not suitable, however, for producing a device with fine 3D features such as the fluid channels, so the researchers turned to 3D printing, which allowed them to create the device in one piece. They experimented with several resins, but finally settled on Pro3dure GR-10, which is often used to make mouth guards. The resin is nearly as transparent as glass, can be printed in very high resolution, and has hardly any surface defects – and it doesn’t harm the cells.
“When you print some of these other resin materials, they emit chemicals that mess with cells and kill them. But this doesn’t do that,” Velasquez-Garcia said. “To the best of my knowledge, there’s no other printable material that comes close to this degree of inertness. It’s as if the material isn’t there.”
The device also features a “bubble trap” and a “tumor trap.” Fluids flowing into a device like this one creates bubbles that can disrupt the experiment or burst and release air that destroys tumor tissue. So the researchers created a bubble trap, a chimney that rises from the fluid channel into a threaded port through which air escapes. Fluid gets injected into an inlet port adjacent to the trap, then flows past the trap, where any bubbles in the fluid rise up through the threaded port and out of the device. Fluid is then routed around a small U-turn into the tumor’s chamber, where it flows through and around the tumor fragment.
The tumor trap sits at the intersection of the larger inlet channel and four smaller inlet channels. Tumor fragments, less than one millimeter across, are injected into the inlet channel via the bubble trap. As the fluid flows through the device, the tumor is guided downstream to the tumor trap, where it gets caught. The fluid continues traveling along the outlet channels, which are too small for the tumor to fit into, and drains out of the device. A continuous flow of fluids keeps the tumor fragment in place and constantly replenishes nutrients for the cells.
“Because our device is 3-D printed, we were able to make the geometries we wanted, in the materials we wanted, to achieve the performance we wanted, instead of compromising between what was designed and what could be implemented — which typically happens when using standard microfabrication,” Velásquez-García said.
The next step is to test how the tumor fragments respond to therapeutics.
“The traditional PDMS can’t make the structures you need for this in vitro environment that can keep tumor fragments alive for a considerable period of time,” said Roger Howe, a professor of electrical engineering at Stanford University, who was not involved in the research. “That you can now make very complex fluidic chambers that will allow more realistic environments for testing out various drugs on tumors quickly, and potentially in clinical settings, is a major contribution.”
Discuss this and other 3D printing topics at 3DPrintBoard.com or share your thoughts below.
[Source/Images: MIT]
Paul Benning Chief Technologist 3D Printing at HP Predicts 3D Printing Developments in 2019
Paul Benning is the 3D Print and Microfluidics Chief Technologist at HP. Before that he was an HP Fellow and the Chief Technologist of their imaging and printing division. He’s a noted expert in nanotech, microfluidics, and inkjet with a Ph.D. in Material Science. I’m really impressed with the caliber of people at HP and the amount of thought that they put into their technology and all of the aspects that surround their technology. I, therefore, jumped at the chance to interview Paul about some key trends for 2019. I’m glad that we got to learn that HP is able to print circuits on its machines and that they’re going to be incorporating machine learning into manufacturing. I’m also glad that Paul’s predictions are firmly rooted in practice science and manufacturing and not in “dream a little dream” blah blah like so many predictions.
Machine learning could significantly reduce scrap rates in 3D printing, is this something you would expect to happen in the near term?
I expect machine learning to escalate innovation in the manufacturing industry in the coming year. As machine learning is integrated more into 3D printers and control systems around the world, engineers and designers will be able to receive information about the temperature of the machine, what the powder looks like, binding agents used, image data and final part geometries. All of this information will aid in reducing scrap rates and ensure the parts produced are concise and fully functional.
How do you see machine learning and 3D printing interact?
In addition to being able to share more information than ever before with engineers and designers, the integration of machine learning and 3D printing delivers the capability to monitor a part in the field. People can follow the finished part into the world and see how it performs over its lifespan, tying the findings back to design configurations. Designers can uniquely peg every party and track them via a serial number, enabling real-time supply chain traceability.
Can machine learning be used to reduce part shrinkage for example?
Yes, machine learning will be used to improve the process during development and to provide real-time feedback during part printing and this level of control will help to optimize all performance vectors including part shrink. An even more significant impact of machine learning might be in more precisely predicting part shrink so that the design and process can pre-compensate for the predicted deformation producing a printed part with tighter tolerances.
Do you really think that generative design will make designers faster? How?
Absolutely. Generative design has the potential to make designers 10-100x faster by leveraging algorithms to discover every possible iteration of a solution. Engineers can create and simulate thousands of designs – including those that they’re unable to envision themselves – in a fraction of the time. The beauty of generative design is that engineers are no longer limited by their own imagination but can instead leverage artificial intelligence to co-create better products in a faster and more sustainable fashion.
To what extent will simulation improve generative design so that parts can be optimized?
By building in simulation and testing to the design process, engineers avoid expensive manufacturing re-works. This helps optimize parts by ensuring technology is an active participant in the design process, rather than simply reflecting actual finished designs.
Do you also expect toolpath optimization for particular geometries?
For Multi Jet Fusion and Metal Jet the “toolpath” is replaced by the printed pattern and decisions of where drops of each agent are placed. I do expect that the drop placement patterns will be optimized for particular geometries to produce precise and mechanically strong parts.
And if we do how do we feed this information back into generative design software?
Using information shared back from machine learning monitoring 3D printed parts in the field, designers and engineers can input real parameters into generative design software, such as product size or geometric dimensions, operating conditions, target weight, materials, manufacturing methods and CPU. The software then generates all the feasible designs and runs a performance analysis for each to determine the best ones for prototyping.
I’ve always found it rather tantalizing that with the HP 3D printing technology you could put lots of coatings on objects. With porosity and surface quality being so problematic for us, is coating parts something that you’re looking at?
Yes, we are investigating coatings that are applied both as a post-print process and during print in multi-agent systems. This voxel control capability will allow HP 3D printers to go beyond simple coatings to produce patterned surfaces – different coatings in different locations – and even enable materials control away from the surface, inside the part.
Can we expect circuits and conductive materials from HP 3D printing?
We have demonstrated printed circuits at HP Labs and shown operational sensors like strain gauges.
With binder jetting metals won’t we always have a problem with part shrinkage? And won’t that always be terrible because shrinkage will vary due to part geometry and size?
In any technology where we start with powders and create dense final parts there will be shrinkage as the open space between powder particles is consumed. We can produce some quite complex geometries today and I expect that as we continue to learn our models will improve and we will provide design tools that work with predictable shrinkage and clever support strategies to provide the broadest design space.
How can you ensure that these parts have the right tolerances?
Well-characterized production machines that are operated using good process control methodologies will give high confidence in producing parts with the expected tolerances. Advanced computational modeling and machine learning will help us automate the design process to get the best design quickly and will help automate the production tool setup and control processes so we build the best part every time.
What are some application areas for 3D printing that you see opening up?
We’ll see accelerated impact of digital manufacturing take hold in the form of production applications, particularly across the automotive, industrial and medical sectors. In the auto sector, we’ve seen an increased focus on developing production-grade materials for auto applications as 3D printing gravitates from prototyping to full production of final parts and products. Additionally, as new platforms such as electric vehicles enter mass production, HP Metal Jet is expected to be leveraged for applications such as the light weighting of fully safety-certified metal parts. Industrial 3D manufacturing also enables the automotive industry to produce applications in new ways that were previously impossible, along with the ability to design application-specific parts for individual systems or models.